1 Cell biology review
- Overview of cell biology
- Inside the cell:
- Cell membrane, cytoplasm
- Organelles
- Outside the cell:
- Ground substance
- Fibers
- Cell processes:
- Cell division
- Cell death
- Cell contacts
- Signal transduction
- Summary

Overview of cell biology
The cell is the basic unit of life. It is the smallest thing that we call living (without arguing about viruses), and the human body is made of 30 trillion of them. We started off as a single cell, and the purpose of this class to learn a little bit about how that one cell developed into the trillion-celled organism you are today. Nearly all of the instructions for making trillions of cells– how to make them, when to make them, and where to make them– are found within every single cell. That means cells in your teeth have the instructions for making toes. One important concept in this chapter is to see things in the context of nature versus nurture. When we discuss how a cell’s instructions influence the health of a patient, that is an example of nature.
Different cells have different functions, but every cell in one person has the same instructions (with a few exceptions). To become different from one another, cells use those instructions differently. This is an important process called differentiation←. In a nutshell, this is no more complicated than the process of boring cells becoming different from one another. Differentiation is guided by a cell’s environment, which we say is an example of nurture.
We give different names to cells as they make changes. We always start with some flavor of stem cell. If that stem cell is able to become, oh let’s say a smurf, that stem cell is usually named a Smurf Stem Cell. As the Smurf Stem Cell divides and differentiates into cells that build a smurf, those builders are named Smurfo-blasts. When that smurf is finished, the cells inside the smurf are named Smurfo-cytes. Would you prefer real names? The stem cells found in a tissue called mesenchyme← are named mesenchymal stem cells, the cells that make dentin are named odontoblasts, and the cells in cementum are named cementocytes (stem to blast to cyte). Differentiation is not a single step, but a series of steps along a spectrum. We start from the least differentiated cells, to more differentiated cells, until we reach a terminally differentiated cell. The aim of this chapter is to review the parts of a cell that help us to explain difficult concepts like differentiation and development. This textbook assumes you’ve been introduced to cell biology before, therefore we cover the parts of the cells briefly. Also, we focus our coverage to cell parts that play important roles in tooth development, while skipping the rest.
One more important concept introduced in this chapter may seem simple, but it is deceptively complicated: the difference between a cell and Extra-Cellular Matrix(ECM). There are cells, and there is the stuff between cells– it’s so simple! Enamel and dentin are ECM. That ECM is made by cells. Those cells mostly reside within the pulp cavity. It will be your task to understand how those cells make the ECM, and understand what the ECM is. The periodontium and oral mucosa are a mixture of cells and ECM. In this chapter, be sure you learn and understand what a cell is versus what ECM is before we get to these interesting tissues.
If you need more than a short refresher in cell biology, here are links from the National Institute of Health (NIH), who have a number of useful publications and videos (for free), including:
3D animations of the human cell
- WEHI tv (relaxing animations of DNA and more)
Learn genetics (interactive website)
Inside the Cell (pdf and epub downloads)
Two useful (also free) eBooks you may wish to download are from OpenStax. Sometimes you just need to go to your big reference eBook and search for the term you know you learned about years ago (cntrl-f can save you loads of scrolling)

Inside the cell
Cell membrane and cytoplasm
Every human cell is surrounded by the plasma membrane. In this book, we focus on materials that are on the inside of this membrane, on the outside of this membrane, and in the membrane itself. The plasma membrane separates the cell from its environment, and allows certain materials to enter or leave. Phospholipids and cholesterol form a lipid bilayer, creating a membrane. The double-sheet of lipid molecules acts as a barrier, separating the cell from the external environment. The other major component of the plasma membrane are proteins. Trans-membrane proteins span the plasma membrane. Some trans-membrane proteins regulate what goes in or out of the cell. Other trans-membrane proteins receive signals from other cells or the environment, and relay that information to the inside of the cell. Still others trans-membrane proteins mediate attachment to structures outside of the cell. Not all membrane proteins span the entire width of the phospholipid bilayer, and are located on the outer or inner surface of the plasma membrane.
Many biology classes focus heavily on the nucleus. That’s fine. But one of the first African Americans to earn a Ph.D., Dr. Ernest Everett Just, in his book Biology of the Cell Surface
, wanted scientists to look more closely at the plasma membrane. Dr. Just was an embryologist, and in embryology the plasma membrane proteins, especially receptors and cell adhesion molecules, are very important. Receptor proteins can guide cells through the steps of differentiation that ultimately lead to the development of human morphology. Cell adhesion molecules adjust the integrity and permeability of a tissue, leading to relatively higher permability of junctional epithelium← compared to the epidermis of the skin.
Inside the plasma membrane is cytoplasm. Cytoplasm is the filling of a cell, sometimes referred to as Intra-Cellular Fluid (ICF). Cytoplasm includes nutrients and electrolytes absorbed from the the fluid surrounding cells. In addition, the cytoplasm includes numerous proteins and glycoproteins synthesized by the cell. These molecules have other important functions, but they attract water from the ECF. The end result is cells have a gelatinous filling, rather than a watery one. This gelatinous filling is filled with a number of organelles, similar to the way grandmother’s jello salad contains grapes, raisins and food-like substances that do not in any way turn jello into an actual salad.
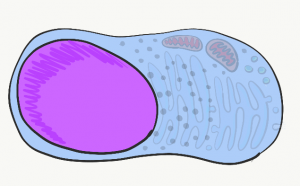
The nucleus
The nucleus contains practically all of a cell’s Deoxyribonucleic acid (DNA). DNA is the instructions for making a Ribonucleic Acid (RNA) copy, a process known as gene transcription. Most RNA is translated in the cytoplasm into proteins found inside and outside of a cell. In addition to instructing a cell what protein to make, DNA instructs when and where to make proteins. For instance, epithelial cells of the oral mucosa do not make protein enzymes that secrete calcium and phosphate into ECM. On the other hand, epithelial cells that differentiate← into ameloblasts do make these proteins. They do so by expressing the DNA for these enzymes, after being told to do so by neural crest cells←. All cells in the human body have the same DNA (with a few exceptions), which means oral mucosa cells could secrete calcium and phosphate. However, different cells express different DNA at different times.
DNA can be divided into 2 basic regions. There are genes, each gene is more-or-less the instructions for a single protein. Genes are read in a linear fashion (like you are doing now). The rest of DNA folds up into 3-D shapes that provide instructions for when and where to express genes. These 3-D shapes are referred to as non-coding DNA
. If geses are like pages of a book with words on them, non-coding DNA looks more like origami and acts more like a substrate for an active site (hopefully you are familiar with enzyme kinetics
). To make a protein using the instructions in a gene, other proteins called transcription factors bind to non-coding regions of DNA. From there, they unzip the nearby gene, and recruit enzymes that transcribe one strand of DNA into messenger RNA (mRNA). The mRNA then leaves the nucleus to be translated into protein. Not all transcription factors activate gene transcription. Proteins called repressors
inhibit the expression of genes, too.

Transcription factors turn genes on and off quickly. These on/off switches can respond to changes in a cell’s environment. But when cells differentiate←, they shut down genes they won’t need more permanently. Rather than allow transcription factors to act like on and off switches, unnecessary genes are methylated ( -CH3 groups are attached to the DNA), packed up around histone proteins, and kept in long-term storage. You will read about this repeatedly throughout this book: the pattern of DNA methylation and histone packaging is copied during mitosis. This means the pattern of genes that are available or packaged away is inherited by both daughter cells. Because this inheritance is not a difference in the DNA sequence, it is known as epigenetic inheritance. Epigenetic traits play an important role in cell differentiation← and cell fate, covered in more detail in chapters 6 through 11 (further reading on epigenetic traits)
.
We have 46 molecules of DNA in the nucleus– they are very long molecules, but only 46 in number– 23 maternal, and 23 paternal. During mitosis, these 46 molecules are duplicated and packaged up tightly into 92 chromosomes. This packaging involves re-using histones along nearly the entire length of a DNA strand, and allows chromosomes to be seen under the light microscope. The rest of the time, DNA is mostly unwound (un-needed instructions are wound around histones, the rest wiggles around randomly, free to be transcribed if instructed). This unwound DNA fills up the nucleus in a way that doesn’t look very exciting. We call that DNA chromatin, it is functionally it is the more exciting of the two forms.
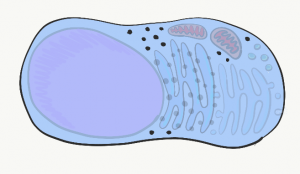
Ribosomes
Visible throughout the cytoplasm are small specks made of protein and RNA called ribosomes. These structures translate mRNA instructions that come from the nucleus. mRNA instructions guide the linkage of amino acids into a long protein. Groups of three mRNA nucleotides, called codons, instruct the ribosome which amino acid to add next to the protein. Like regulatory regions of DNA, ribosomal RNA (rRNA) isn’t read linearly but folds into a 3-D shape. That shape, along with ribosomal proteins, create active sites that catalyze the chemical reaction of mRNA translation. Free-floating ribosomes in the cytoplasm synthesize proteins that remain in the cytoplasm, such as keratin or enzymes that mediate apoptosis. If our description of gene expression left you wondering “if proteins convert DNA instructions into protein, where did the first proteins come from?” we have some reading material on RNA enzymes (and the beginning of life) here
.
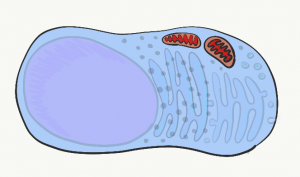
Mitochondria
The mitochondria are where the majority of Adenosine Tri-Phosphate (ATP) is produced. ATP is made of Adenosine,
plus three phosphate
(PO43-) groups– pay attention to the phosphate part, it is also a major component of bone, enamel, dentin and cementum. ATP powers almost all cellular processes, including the transcription and translation of mucous proteins within a salivary gland, the electrical signals sent by neurons in the tongue when food enters the oral cavity, and the contraction of myo-epithelial cells to cause salivation. Mitochondria burn glucose, using oxygen, and harness some of the energy released in the form of ATP. Mitochondria are different from other organelles in that they contain a little bit of their own DNA, which is inherited just from mother. Mitochondria contain two phospholipid bilayer membranes, versus one like other organelles. This extra membrane is used during ATP synthesis. Perhaps you covered glycolysis
and the citric acid cycle
before. The part to remember is that mitochondria use a proton (H+) gradient, which makes the inside of mitochondria acidic. It is toxic to the rest of the cell should mitochondrial membranes become damaged.
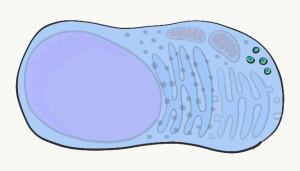
Lysosomes
Lysosomes are small compartments surrounded by a phospholipid bilayer (a bilayer similar to plasma membrane). Inside lysosomes are acids and digestive enzymes. They are used to destroy stuff inside the cell when it wears out, or materials the cell phagocytosed
from outside (e.g. debris, bacteria). When a cell dies and begins to break apart, neighboring cells are in danger of being damaged from the acids and enzymes found within lysosomes. In the oral cavity, epithelial cells only have a life-span of days before they wear out, so it is very important for these cells to neutralize acids and enzymes in their lysosomes before dying (part of a process called apoptosis).
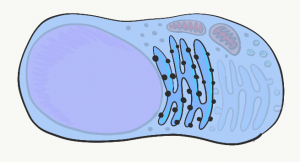
Endoplasmic Reticulum
The Endoplasmic Reticulum (ER) is a series of interconnected tubes surrounded by a phospholipid bilayer– similar to lysosomes, only bigger, more tubular, and not full of acid. The smooth Endoplasmic Reticulum (sER) is where cells produce lipids and store calcium. The rough Endoplasmic Reticulum (rER) (Fig. 1.8) is covered in ribosomes. Proteins made by these ribosomes wind up inside the rER, travel to the Golgi apparatus, and are secreted (such as proteins in mucus) or stay within the plasma membrane (such as cell-junction proteins, or receptors for morphogen← molecules).
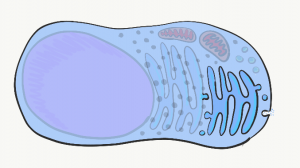
Golgi apparatus
The Golgi apparatus is another set of tubes, similar to the rER. Small membrane-enclosed spheres called vesicles shuttle proteins made in the rER to the Golgi apparatus, where the proteins are modified. Often, these proteins have sugars attached to them, making them glycoproteins. New vesicles take these proteins to the plasma membrane, where they are either secreted or become a part of the plasma membrane. An example of a secreted protein is collagen, found in cementum, dentin and the PDL. Other secreted proteins include the glycoprotein fibronectin and the trans-membrane protein integrin, which play important roles in the healing of damaged gingival tissue.

Fluorescent cells
by NIH image is in the Public Domain CC0Cytoskeleton
The cytoskeleton is a structural network within the cytoplasm. The cytoskeleton is composed of long structural protein fibers. Shown in Fig 1.10, cells have microtubules and actin filaments stained red and green, respectively. You won’t see this on old-fashioned histology images– both proteins stain pink on a H&E stain, as do soluble proteins in the cytoplasm. Motor proteins
, such as myosin and dynein, bind to cytoskeleton fibers and move. Depending on what the fiber and motor proteins are anchored to, this can cause the cell to change shape, to migrate, or move materials within the cell (such as vesicles or chromosomes). During dentinogenesis←, it is critical for odontoblasts to secrete enzymes that catalyze mineralization of dentin from only one side of the cell (from the odontoblastic process). Otherwise, odontoblasts could become cemented within their own secretions. This involves moving vesicles along the cytoskeleton, toward the apical surface of the cell. It is also important for mesenchymal stem cells to migrate over protein scaffolding during would repair in the oral mucosa. This involves changing the length of cytoskeleton proteins, which changes the cell’s shape. Shortening the cell while extending the next pseudopod pulls mesenchymal stem cells along fibers in the ECM.
Outside the cell
Extra-cellular matrix: | Description: |
---|---|
Ground substance | The background color |
Fibers | Lines over the background |
Extra-Cellular Matrix (ECM) includes all the material found outside of cells. It is usually divided into the two parts listed in Table 1.1.
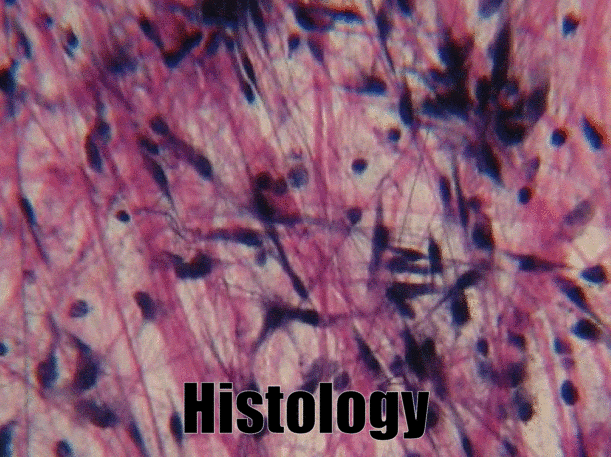
Ground substance
Ground substance is the name for material outside of the cell that has no particular shape when viewed under traditional microscopy. One part of ground substance is Extra-Cellular Fluid (ECF), which is the water and nutrients surrounding cells. This fluid is plasma when it is inside of a blood vessel, but once this fluid exits the blood and surrounds cells, it is called ECF. This fluid does not flow or drip, it is held in place by large solutes found in ground substance, including proteins, glycoproteins, and polysaccharides
. Small solutes tend to diffuse away, down their concentration gradient. The large molecules of ground substance are stationary, and instead hold fluid in place, forming a gel. Ground substance doesn’t look like much under a microscope, no more than if you looked really closely at jello. These proteins, glycoproteins and polysaccharides are made and secreted by cells (these cells have a lot of rER and Golgi apparatus).
One of the glycoproteins found in ground substance is fibronectin. Cells may recognize, bind to and move along fibronectin if they have the correct integrin (a trans-membrane protein) spanning their plasma membrane. Fibronectin acts not only as a road along which cells travel, it is also a road map. Getting the right cells to the right place at the right time is very important both in wound healing and in development. In fact, what you learn about development is re-used (recapitulated) in wound healing.
Another important molecule found in ground substance is a large polysaccharide called hyaluronic acid. Like fibronectin, cells bind to and travel over hyaluronic acid (using a different type of plasma membrane protein). Hyaluronic acid has applications in dentistry
, such as helping cells of the gingiva stick to a dental implant to form a bacteria-resistant seal. We can’t see fibronectin or hyaluronic acid without using modern imaging tricks, which is why they are listed as a part of ground substance, and not in the next section, fibers. Because cells migrate over ground substance proteins, we say that ECM proteins function as a scaffold. Without scaffolds, tissues grow only from their edges. This is fine if speed is not critical, such as when enamel and dentin are forming, while the embryo is safe inside a uterus. Following an injury, however, it is optimal for a wound to heal everywhere at once, not just from the edges. The body often puts down some form of scaffolding first, such as a scab. In dentistry, artificial scaffolds help the body heal faster, reducing the need for surgical replacements. Examples of artificial scaffolds include some bone grafting← materials and periodontal membranes←.
In addition to their structural role guiding cells to new locations, ground substance molecules provide cells with positional information. This information tells cells where they are located, and what they should be doing. For instance, when a stem cell binds to fibronectin, fibronectin can instruct the stem cell
to express different genes and differentiate← into a specific type of cell. This can mean the difference between a stem cell differentiating into an odontoblast which creates reactionary dentin, or a fibroblast which creates scar tissue. Getting cells to the correct location is nice, but they need to know what to do when they get there. When we cover tooth formation and tooth repair, remember these functions of fibronectin and hyaluronic acid.
As we learn more about how ground substance instructs stem cells, we get better at helping teeth and periodontal tissues to repair themselves.
Fibers
Three stringy shapes were visible under a light microscope a century ago, and were grouped together as fibers of the ECM. Like fibronectin and other ground-substance molecules, fibers are secreted by cells called fibroblasts.

Collagen is the strongest of the three fibers. It is therefore referred to as a structural protein—it gives many organs their shape. Collagen is a large protein. Getting collagen from the rER (where it is translated) to the Golgi apparatus where it is modified, and finally secreted from the cell requires extra-large vesicles
for transport. Collagen fibers are made of 3 coiled α-helices
, that are in turn coiled and cross-linked together, making a very strong macromolecule with the same basic shape as a rope. It is very strong if you pull on it from the ends, but bends if you apply force from the side. For instance, an area of advanced caries on a tooth can be as soft as wet driftwood. It contains collagen fibers without the plentiful minerals that used to surround them. Collagen fibers are found in regions of the oral cavity where the ability to resist force is important, such as in dentin and the PDL. In fact, collagen is found throughout the human body, accounting for 25% of our protein content.
In addition to having a structural role, collagen acts as a scaffold. Similar to fibronectin and hyaluronic acid, cells use collagen fibers to migrate along. Like fibronectin, collagen also provides cells with positional information. This is possible because there are 28 different collagen genes which are assembled into slightly different versions in different locations (similarly, fibronectin comes in multiple isoforms thanks to alternative splicing
). For example, IEE cells differentiate into ameloblasts after they come into contact with type IV collagen found in pre-dentin, rather than type I found in the basement membrane.
Reticular fibers, which are not shown in Fig. 1.11, look like a fine, spider web-like mesh under the microscope. Long after histologists first saw and named reticular fibers, molecular biologists discovered that reticular fibers are made of the collagen protein. Nevertheless, they have their own name, and receive equal footing with collagen fibers on the list of ECM fibers. This web-like network of protein isn’t as strong as collagen fibers, but provides enough of a frame for blood cells to rest in organs like the spleen and lymph nodes. Without reticular fibers, loose cells would sink to the bottom. To summarize: collagen proteins can form collagen fibers, or collagen proteins can form other shapes that aren’t fibers, including reticular fibers. Got that? We apologize. Categorizing molecules as ground substance versus fibers is based on what things look like under a microscope. The collagen protein is a single molecule that is transcribed and translated from a gene. This won’t be the last time where the lineage (in this case, genes) does not match the morphology (physical appearance). Be prepared to face this question repeatedly: which is more important, lineage or appearance?
Elastic fibers are thinner than collagen fibers, and look like fine hairs under the microscope. They are made from a different protein (elastin
). As their name suggests, elastic fibers can be stretched and spring back to their original length. This is not something collagen does well. If you can’t put your palms on the floor while keeping your legs straight, the collagen fibers of your hamstring and gastrocnemius tendons are limiting your range of motion. Regular stretching exercises activate fibroblasts, which in turn lengthen collagen fibers. Elastic fibers are found in higher quantity in regions of the oral cavity that change shape during speech or swallowing, such as the soft palate.
Cell processes:

Far-Red & Near-infrared Fluorescent Ubiquitination-based Cell Cycle Indicator (FUCCI")
by Erin Rod is licensed under CC BY-SA 4.0 / converted to gifMitosis
Cell division, or mitosis, is the process by which one cell replaces itself with two copies. During mitosis, one cell divides into two identical daughter cells– there is no parent cell left after cell division. During development, we grow from a single cell into 30 trillion cells, so a lot of mitosis occurs. In the time it takes you to read this sentence, several billion cells in your body will go through mitosis to replace cells that just died. When a cell is not undergoing mitosis, it is said to be in interphase
(in Fig. 1.15, interphase is G1, S and G2 combined). This is the time where a cell might be doing its job, such as secreting fibers or preparing for mitosis. Before mitosis can occur, a cell must have roughly double of everything. During mitosis, everything is divided in half between two new daughter cells.
Not all cells are capable of mitosis– in fact, most cells in an adult have differentiated←. These cells are performing tasks, they are too busy to reproduce. We say these terminally differentiated cells have exited the cell cycle.
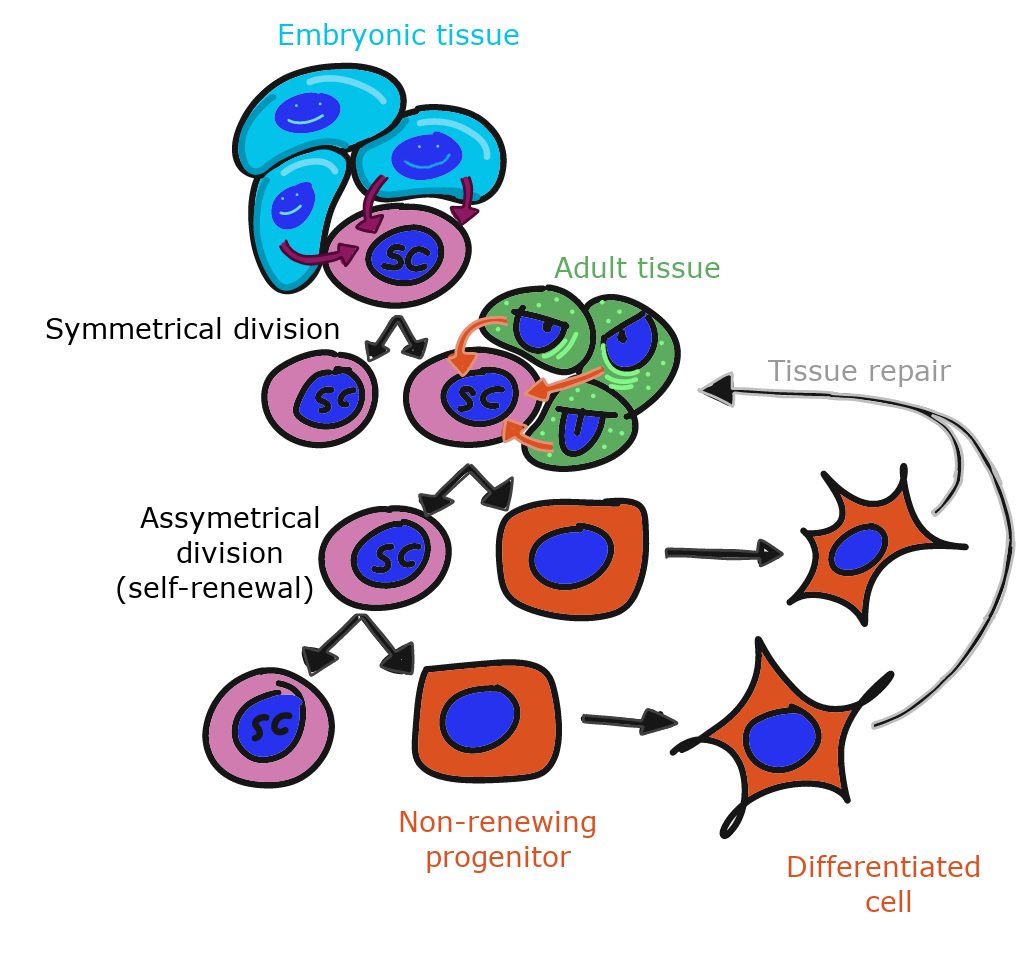
To repair damage, tissues have stem cells. A stem cells is an undifferentiated cells that is capable of dividing and differentiating into one or more different types of cells. When an adult stem cell divides into two daughter cells, one daughter typically remains a stem cell, and the other differentiates into something else. A tissue therefore keeps a steady supply of stem cells, as long as the stem cells don’t die before they undergo mitosis. As we get older, our tissues don’t heal as well because some stem cells have died before they replaced themselves. After early development, when a stem cell dies, it is gone. Another stem cell does not undergo mitosis to produce two stem cells to replace it.
Stem cells are named based on how many different types of cells they can potentially become. The uni-potent stem cells of the oral epithelium become keratinocytes, and only keratinocytes. The multi-potent neuro-mesenchymal stem cells differentiate into odontoblasts, cementoblasts, fibroblasts and osteoblasts (and might be referred to as partially differentiated). The omni-potent fertilized egg becomes every cell in a human, plus more.

"Anatomy and Physiology Fig 18
” by OpenStax is licensed under CC BY SA 4.0To go through interphase and prepare for another round of mitosis, cells go through a series of cell cycle checkpoints. Checkpoints set up the base rate for cell division, which helps ensure that the correct amount of tissue growth occurs. This is like an hourglass, only instead of grains of sand accumulating in the base, it is phosphate residues accumulating on cyclin proteins
. Cyclins are transcription factors that activate genes that allow progression through the checkpoint. Their activity is regulated by phosphorylation (carried out by enzymes called Cyclin-Dependent Kinases, or CDKs). The speed of this process can be sped up or slowed down by external signals, such as growth factors. Growth factors are hormones that are secreted into the ground substance of a tissue. The density and stickiness of the ground substance influences how far the growth factor diffuses. If diffusion is limited, the growth factor only speeds up cell division in a localized area. This is important in the formation of new organs such as teeth, and for tissues such as bone and PDL fibers. Other growth factors might spread over a wide area, especially if they are secreted into the bloodstream. Secretion of Growth Hormone into the blood, for instance, allows different organs to grow at roughly the same speed.
Mutation in a gene for a growth factor, or the receptor for a growth factor, can lead to a gain-of-function (more reading here)
. If a mutation to a gene causes a cell to receive a constant go-signal for passage through the cell cycle, we call the mutated gene an oncogene
. In this book, we cover the role of Wnts in head and tooth development, but Wnts were first discovered for because they cause cancer. It takes just one mutated copy of an oncogene to gain a function. Some mutations can stop proteins from functioning. If those proteins are necessary for passage through the cell cycle, we call those tumor suppressor genes
. One example of a tumor suppressor gene is Retinoblastoma protein (pRb), which normally halts progression from G1 to S phase. For a cell to lose the ability to stop at a cell cycle checkpoint, both copies of a tumor suppressor gene alleles must be mutated
. A metaphor might be helpful here. In your car, it takes one foot to step on the gas too hard, but you would have to be missing two feet to not be able to hit the brakes (loss-of-function). Usually, cancers form when a single cell acquires mutations to multiple oncogenes and multiple pairs of tumor suppressor gene alleles. An allele
is one of the two copies of a gene found in the same position on two homologous chromosomes. Even when all of these mutations happen in a single cell, there is another layer of protection covered in the next section.

"43705"
by Birgite Janicke is in the Public Domain CC0 / converted to gifApoptosis
All cells contain a group of cell-surface receptor proteins and intra-cellular enzymes that allow them to undergo programmed cell death
, or apoptosis. Programmed cell death is critical to multi-cellular life. read that again, it is an odd thing to say. Without programmed cell death, our bodies would quickly fill up with old, non-functioning cells. Even worse, when cells die– the lifespan for epithelial and blood cells is only weeks– they release the contents of their lysosomes and mitochondria. The contents of these organelles are acidic and contain digestive enzymes, which damage or kill neighboring cells. If those neighboring cells die as a result, they too would release their lysosomal and mitochondrial contents, causing even more damage. When this happens in the human body, it is called tissue necrosis. That’s not all, a dead cell spews out DNA, and DNA is long, stringy and sticky. This can trap other cells and prevent them from migrating properly. Spewing out DNA is great at trapping and killing bacteria
, assuming the spewing cell doesn’t mind dying in the process. But this is not something good to do to neighboring human cells on accident. This is related to how a periodontal infection can lead to destruction of the periodontium, but doesn’t directly cause the destruction.
To avoid the postitive feedback loop of cell death leading to more cell death, cells can be instructed to undergo apoptosis. This occurs as cells reach the end of their lifespan, if the immune system has determined cells to be infected or cancerous, or if cells aren’t needed anymore. Apoptosis ensures that before a cell dies, it neutralizes the pH of its lysosomes and mitochondria, and chops up its DNA into safe, small bits.
Apoptosis is especially important during embryonic development. During development, more cells are produced than needed. Later, the extra cells are removed in an organized fashion. This is similar to the way construction of a large building involves building scaffolding first, and removing the scaffolding towards the end of the project. The process of wound repair also involves an over-production of cells followed by their organized removal. Hopefully, this will seem logical by the time you finish this book. During wound repair, DNA instructions are turned on again that were last used during embryonic development. If you want to sound fancy, and don’t we all, you can say wound healing recapitulates embryonic development
. To recapitulate means to state again (repeat).
The process of apoptosis begins either with an internal or an external signal. Internal signals include when a cell’s DNA becomes too mutated, or if there are an odd number of chromosomes during mitosis. Alternatively, a cell can be instructed to undergo apoptosis from an extracellular signal (a cytokine) such as RANKL (a member of the Tumor Necrosis Factor (TNF) family of signaling molecules)
. RANKL activates a trans-membrane protein receptor, which in turn activates a series of enzymes called caspases, which leads to the neutralization of acids, destruction of DNA, and cause the cell to explode into numerous small bits (Fig. 1.15). These bits can be easily cleaned up by macrophages (which are stimulated by RANKL when other cells begin apoptosis).
Cell junctions
Types of cell junctions | Examples |
---|---|
Cell-to-cell | Desmosomes, tight junctions, gap junctions, Cell Adhesion Molecules. |
Cell-to-ECM | Hemi-desmosomes, Cell Adhesion Molecules. |
Junctions are specialized groups of proteins on or near the cell surface that make connections to other structures. These connections can be to other cells, or to the ECM, as listed in Table 1.2. Cell-to-ECM contacts are very important for cell migration. Cell-to-cell contacts allow receptors on the cell surface of one cell to bind to molecules on another cell, which is the basis for most cellular communication in very early development.

A desmosome, also known as macula adherens
by Mariana Ruiz LadyofHats is in the Public Domain CC0Desmosomes
Cell-to-cell junctions called desmosomes (anchoring junctions) are strong connections between two cells. Desmosome proteins pair up and anchor the cytoskeleton of one cell to its neighbor. A large group of cells anchored together by these junctions are much stronger than the sum of the individuals.
“A single twig breaks, but the bundle of twigs is strong” —Tecumseh
Hemi-desmosomes are half of a desmosome anchored to the ECM, such as the seal between the junctional epithelium and the non-cellular surface of a tooth. One of the many proteins in a desmosome is an integrin. This protein recognizes and binds to proteins in the ECM such as fibronectin. When the integrin protein of a cell connects to fibronectin, this does two major things. First, it anchors the cell’s cytoskeleton to the ECM and anchor the cell in place. Integrins also signal to the inside of the cell, allowing the nucleus to know what type of tissue the cell is located in.
Before a cell can migrate to a new location, it must first remove its desmosomes. During development, cells frequently migrate to new locations and form new structures. During wound healing, stem cells detach from their neighbors, migrate into the injured area, and undergo mitosis to create more cells to repair or replace damaged tissue.

Diagram showing a tight junction
by Mariana Ruiz LadyofHats is in the Public Domain CC0Tight junctions
Tight junctions are smaller junctions between cells. A ring of tight junctions completely encircles a cell, and creates a water-tight seal between that cell and another cell. When lots of cells are joined in this fashion it creates barriers between one part of the body and another, allowing the cells to regulate what goes across and what does not. This also gives cells apical-to-basolateral polarity← (or a difference between top and bottom), which is especially important to an epithelium. Proteins synthesized on the rER can be sent to either the apical or basolateral portion of the plasma membrane. Once there, trans-membrane proteins of the apical side of the cell cannot diffuse to the plasma membrane on the basolateral side, because the ring of tight junctions blocks their movement.

the diagram shows a cell union called gap junction
By Mariana Ruiz LadyofHats is in the Public Domain CC0Gap junctions
Gap junctions (or connexons) are a group of proteins that form a small passage between cells, and can be opened or closed. This gap allows cells to communicate directly with one another. Because of the way epithelial cells are connected to each other– in a sheet– this communication occurs across a plane. This is one way that cells know their position relative to one of the body’s axes, and is a process called planar cell polarity (PCP) (further reading on PCP
). Planar cell polarity is side-to-side polarity, while apical-to-basolateral polarity← is top-to-bottom. Planar cell polarity allows cells to know what direction they are facing in the body, ensuring that the structures they are forming are not only in the correct location, but in the correct orientation. For instance, planar cell polarity helps teeth to erupt straight into the oral cavity, rather than at an angle.
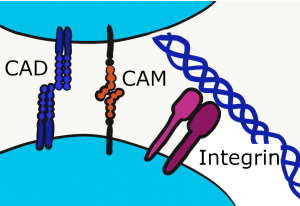
Cell Adhesion Molecules
Cells can stick to other cells (cells of the same type) using proteins not visible under the microscope. Collectively, these are Cell Adhesion Molecules (CAMs), which includes the integrins. These trans-membrane proteins adhere to ECM proteins, or to other CAMs of the same type. For instance, Neuronal CAM (NCAM) only binds to NCAM, not to Endothelial CAM (ECAM). These molecules not only adhere cells to the correct target, they signal to the nucleus when attachements are made, which can trigger differentiation←. Furthermore, it is just as important to let go at times as it is to stick. CAMs regulate the loss-of-attachment necessary for cell migration during important developmental processes like gastrulation←, or later in life during wound healing.

"BMP4 Signal Transduction Pathways"
is licensed under CC BY-SA 3.0Signal Transduction cascades
During development, there is a lot of communication between cells. For two or more cells to communicate, one cell must secrete a signal, such as a growth factor or morphogen← molecule. These signals are usually small molecules that diffuse through the ground substance, or larger molecules that become part of the ground substance. These signals bind to trans-membrane proteins called receptors. The receptor next activate a series of intracellular signals, often enzymes (a signal transduction cascade). During development, these intracellular signals activate or inhibit transcription factors or alter DNA methylation, which in turn changes gene transcription, which in turn changes cell fate. Fig 1.20 illustrates how complex a signal transduction cascade can be. The important concept is that blocking any of the items in Fig 1.20 leads to the same result: the signal will not reach the nucleus, and differentiation← will not occur (loss-of-function). Conversely, chemicals that mimic any of the items in Fig 1.20 trigger differentiation where is shouldn’t be occurring (gain-of-function). We call these chemicals teratogens when they interfere with development.
Later in life, some cells become highly specialized at cell-to-cell communication. Examples include neurons which release neurotransmitters across synapses, endocrine glands which secrete hormones into the bloodstream, and immune cells which secrete cytokines into the bloodstream. You, however, will be reading about less-specialized forms of cell signaling. The signals may be basic, but work perfectly fine for small organisms like embryos. Plus, there needs to be signaling during embryonic development to guide the formation of synapses and the circulatory system.
Summary
Two big concepts introduced in this chapter are differentiation, and nature versus nurture. Differences between two people may be caused by differences in DNA (nature). But not always,. People are different because of their environment as well (nurture). To explain why one patient is healthy and the other is unhealthy you must consider both nature and nurture. Nurture often plays a bigger role. For example, poverty has a much bigger impact on human health
than genetics.
But what about when we discuss different cells in the same person? When we do, nature doesn’t factor in at all (cells have the same DNA). So any differences between two cells must be due to nurture. We don’t mean the environment the patient is in, such as wealth versus poverty. We mean the environment immediately surrounding a cell. We covered many environments in this chapter, including:
- what ECM surrounds the cell
- what signaling molecules (growth factors and morphogens←) are in nearby ground substance
- what receptors and CAMs are on the plasma membrane of the cell
- which genes can be expressed, versus which ones are in storage using methylation and histones
These environmental factors guide the process of differentiation←. Differentiation makes tissues look different in chapters 2 through 5. It makes body structures develop differently in chaptes 6 through 11. But there are similarities as well. Those similarities can be due to cells having the same DNA (nature) or sharing a similar environment (nurture).
< Chapter 0 * navigation * Chapter 2 >
Review questions
In the phrase nature vs nurture, nature refers to genetics (heritable traits).
In the phrase nature vs nurture, nurture means environmental factors that occur after fertilization, not DNA inherited from our parents.
When one cell begins to look different from another. This process involves limiting cell fate by altering gene transcription to become more specialized.
Undifferentiated or partially differentiated cells that can differentiate into various cell types, and proliferate to produce more of the same stem cell.
An embryonic tissue composed of undifferentiated mesenchymal stem cells and mucous ground substance.
Multipotent cells of a connective tissue that can differentiate into a variety of cell types, including osteoblasts, fibroblasts, chondrocytes, myoblasts, blood cells and adipocytes.
A cell of neural crest origin that is part of the outer surface of the dental pulp, and whose biological function is the formation of dentin.
Terminally differentiated cell found within mature cementum lacunae.
When a cell has finished its last possible differentiation step and lost the ability to undergo mitosis.
The process by which animals and plants grow and change.
Extra-Cellular Matrix: Materials in the body, but outside of cells. A network of macromolecules, such as fibers, enzymes, and glycoproteins, that provide structural and biochemical support to surrounding cells.
Specialized tissues that surround and support the teeth, including gingival tissue, PDL, cementum and alveolar bone.
The mucous membrane lining the inside of the mouth. It is a stratified squamous epithelium, named the oral epithelium, and an underlying areolar connective tissue named the lamina propria.
The border of every cell, made of a phospholipid bilayer and proteins.
Proteins embedded within the plasma membrane, with parts both the inside and outside of the cell.
A cell-surface, trans-membrane or cytoplasmic protein that binds to (receives) a signaling molecule and transmit the signal further.
Cell Adhesion Molecules: proteins located on the cell surface involved in binding with other cells or with the extracellular matrix.
The shape (or form) of.
Epithelium on the inner wall of the gingiva that attaches to connective tissue of the lamina propria on the basolateral side and to the surface of the tooth on the apical side.
The superficial epithelial layer of the skin, composed of a stratified squamous epithelium.
the gelatinous material within a living cell, excluding organelles.
A protein that has had sugar residues attached to it in the Golgi apparatus and is often secreted.
Extra-Cellular Fluid is the fluid surrounding cells, but not within blood or lymphatic vessels.
deoxyribonucleic acid is the hereditary material in humans and almost all other organisms.
Ribonucleic acid is a nucleic acid present in all living cells. Its principal role is to act as a messenger carrying instructions from DNA for controlling the synthesis of proteins
The process of converting DNA into an mRNA copy
The process of turning the sequence of a mRNA into a sequence of amino acids during protein synthesis
Cells present only during the embryonic period that deposit tooth enamel.
Copying DNA into a functional product, such as a RNA and/or protein. Controlled by the activity of transcription factors binding to gene promoter regions to recruit RNA polymerase.
A temporary group of cells that arise from the embryonic ectoderm, and in turn give rise to a diverse cell lineage—including melanocytes, cranio-facial cartilage and bone, teeth and periodontal tissue, smooth muscle, peripheral and enteric neurons and glia.
A sequence of nucleotides in DNA that encodes the synthesis of a gene product, either RNA or protein.
A protein that controls the rate of transcription of DNA to mRNA, by binding to a specific DNA sequence.
Single-stranded RNA molecule complementary to one strand of DNA of a gene. It leaves the nucleus and moves to the cytoplasm where it instructs the synthesis of a protein.
Methyl groups added to DNA which change the activity of a gene without changing the sequence, typically repressing gene transcription.
Highly basic proteins found in nuclei that compact DNA into a denser form, unavailable for gene transcription.
The process of cell division, where one cell divides into two identical clones (daughter cells).
Heritable DNA modifications that do not change the DNA sequence but do affect gene activity.
The type or types of cell(s) a stem cell can possibly differentiate into in the future, determined by which genes are methylated and stored around histones, or free to be transcribed.
DNA molecule packaged into thread-like structures found during mitosis, visible under a light microscope.
Unwound DNA and histone molecules, available for gene transcription.
A small particle consisting of rRNA and proteins found in large numbers in the cytoplasm. They bind mRNA and tRNA to synthesize proteins.
One of a family of tough fibrous structural proteins, the key material making up hair, nails, calluses, and the outer layer of skin.
Programmed cell death
Membrane-bound organelles that generate most of the chemical energy needed to power a cell's biochemical reactions.
Adenosine Tri-Phosphate, the energy molecule of cells.
An adjective used to describe a gelatinous, slimy substance such as loose ground substance or mucus.
Epithelial cells found in glands, containing smooth muscle actin allowing them to contract and expel secretions of exocrine glands.
Membrane-bound vesicles that contains acids and hydrolytic enzymes that break down many kinds of molecules.
Smooth Endoplasmic Reticulum, an organelle where calcium and lipids are stored.
rough Endoplasmic Reticulum, an organelle where membrane and secreted proteins are translated by bound ribosomes on the surface of the rER.
A membrane-bound organelle that modifies proteins from the rER and packages them up into vesicles, to be sent to lysosomes, the plasma membrane, or secreted from the cell.
A thin gelatinous secretion composed of primarily of glycoproteins and water.
A substance whose non-uniform distribution governs the pattern of tissue development and pattern formation.
A structure inside (or outside) a cell, consisting of liquid enclosed by a lipid bilayer.
The main structural protein in the ECM of connective tissues
Periodontal Ligament, a group of specialized connective tissue fibers that attach a tooth to alveolar bone.
A large glycoprotein found in the ECM which binds to integrin proteins on the cell surface, involved in cell adhesion, growth, migration, and differentiation.
A trans-membrane protein which allows cells to bind to the ECM protein fibronectin, it is involved in cell adhesion, growth, migration, and differentiation.
A dynamic network of interlinking cytoplasmic proteins (mainly actin filaments, intermediate filaments and microtubules), involved in maintaining cell shape, polarity and migration.
Hematoxylin and Eosin stain, typically turns proteins pink and DNA purple.
The formation of dentin.
Arm-like extension of an odontoblast found within dentinal tubules.
The top (or apex) side of a cell or tissue, usually facing the lumen.
Extracellular matrix material involved in the repair of injured and missing tissues, allows stem cells to migrate into the injured area. It is usually replaced during regeneration.
Gel-like substances in the ECM, composed of water held in place by large molecules (proteins, glycoproteins and glycosaminoglycans).
To state again, or to repeat.
A very large glycosaminoglycan distributed widely throughout connective tissue ECM, epithelial, and neural tissues.
An artificial tooth root that is surgically placed into bone tissue.
The ECM proteins elastin and collagen, visible under the light microscope
A medical procedure in which ground up bone tissue or synthetic bone tissue is placed in an injured site to act as a scaffold, promoting the formation of new bone tissue by the patient's own cells.
Procedures attempting to regenerate (as opposed to replace) lost periodontal structures.
In pattern formation, the development of spatial organization in the embryo that results from cells differentiating at specific positions, which requires that the cells have positional values as in a coordinate system
Tertiary dentin formed from a pre-existing odontoblast, contains narrow or filled-in dentinal tubules.
The basic connective tissue cell type capable of secreting ECM components, including fibers and ground substance proteins.
Inner Enamel Epithelium: a layer of columnar cells located next to the dental papilla, these pre-ameloblast cells will differentiate into Ameloblasts which are responsible for secretion of enamel during tooth development.
Newly formed dentin before calcification and maturation.
A thin sheet of extracellular matrix between epithelial tissues and the underlying connective tissue of the lamina propria or dermis.
A form of collagen, forms a web-like meshwork in ECM.
The developmental history of a differentiated cell traced back to the embryonic cell from which it arises.
Thin protein fibers in the ECM, capable of stretching and returning to their original length, made of the protein elastin.
The posterior muscular portion of the roof of the mouth.
A stem cell found in adult tissues (as opposed to embryonic stem cells found in embryos)
The stratified squamous epithelium of the oral mucosa.
A terminally differentiated epithelial cell capable of synthesizing large amounts of the protein keratin within its cytoplasm.
A subset of mesenchymal stem cells derived from neural crest cells rather than mesoderm, or mesoderm-derived mesenchymal stem cells induced by neural crest morphogens to adopt a more neural fate.
Differentiated cell that deposits cementum matrix.
Control proteins and enzymes which ensure proper progression through the cell cycle, regulating the rate of mitosis.
A signaling molecule capable of stimulating cell proliferation, wound healing, and cellular differentiation.
Signaling molecules secreted directly into the blood which travel throughout the body.
A type of mutation in which the altered gene product possesses a new molecular function or a new pattern of gene expression. Gain-of-function mutations are almost always Dominant.
Signaling molecules first identified for their role in carcinogenesis, then for their function in embryonic development, including body axis patterning, cell fate specification, cell proliferation and cell migration.
A mutation that result in the gene product having less or no function (being partially or wholly inactivated).
a variant or different DNA sequence of a gene
A similarity due to shared lineage between a pair of structures or genes in different taxa.
The death of most or all of the cells in an organ or tissue due to disease, injury, or failure of the blood supply.
a broad and loose category of small proteins (~5–20 kDa) important in immune syhstem responses, including chemokines, interferons, interleukins, lymphokines, and tumour necrosis factors, but generally not hormones or growth factors (despite overlap in the terminology). Cytokines are produced by a broad range of cells, including immune cells like macrophages, B lymphocytes, T lymphocytes and mast cells, as well as endothelial cells and fibroblasts.
Receptor activator of nuclear factor kappa-Β ligand, a pro-apoptosis gene expressed in many tissues.
Direct contact between cells allows the receptors on one cell to bind small molecules on the plasma membrane of different cell. In eukaryotes, many of the cells during early development communicate through direct contact.
Trans-membrane structure specialized for cell-to-cell adhesion.
Half of a desmosome, specialized for cell-to-ECM adhesion.
A type of cell-to-cell contact that prevents diffusion between cells.
Top-to-bottom polarity, where the apical side of a cell (or tissue) is different from the basolateral side
The side of a cell or tissue oriented away from the lumen or external surface.
A trans-membrane protein that when paired allows small cytoplasmic molecules to pass through from one cell to another cell.
A polarity axis that organizes cells in the plane (side-to-side) of the tissue.
A phase early in the embryonic development during which the single-layered blastula is reorganized into a multilayered structure known as the gastrula.
When a chemical or physical signal is transmitted through a cell as a series of molecular events, most commonly protein phosphorylation catalyzed by protein kinases, which ultimately results in a cellular response.
Agents or factors which cause malformation of an embryo.