If there is a magical component to life, an argument can surely be made for it being catalysis. Thanks to catalysis, reactions that can take hundreds of years to complete in the uncatalyzed “real world,” occur in seconds in the presence of a catalyst. Chemical catalysts, such as platinum, can speed reactions, but enzymes put chemical catalysts to shame (Figure 4.1).
Figure 4.1 – Rate enhancement for several enzymes Image by Aleia Kim
How can enzymes accelerate reactions to this degree? Chemical reactions follow the universal trend of moving towards lower energy, but they often have an energy barrier in place that must be overcome. The secret to catalytic action is reducing the magnitude of that barrier. When the barrier is lowered, the reaction occurs more quickly.
To develop a fuller understanding of this phenomenon, it is worth taking a moment to discuss the concept of equilibrium.
When a biochemical reaction is at equilibrium the concentrations of reactants and products do not change over time. This does not mean that the reactions have stopped, but rather that the forward reaction and a reverse reactions have reached a point where the forward reaction occurs at the same rate as the reverse. : If you had 8 molecules of A, and 4 of B at the beginning, and 2 molecules of A were converted to B, while 2 molecules of B were simultaneously converted back to A, the number of molecules of A and B would remain unchanged, i.e., the reaction is at equilibrium.
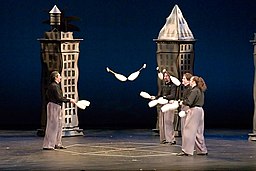
Note that the “equi” part of this word relates to equal, as one might expect, but it does not relate to absolute concentrations.
Biochemical reactions, when not at equilibrium, tend to move in the direction of equilibrium. So chemical reactions involving enzymes are often working in an environment where the concentrations of reactants are shifting, and the flow of reactants to products is influenced by other linked reactions in what is called a biochemical or metabolic pathway.
Activation energy
Figure 4.2 schematically depicts the energy changes that occur during the progression of a simple reaction. In order for the reaction to proceed, an activation energy must be overcome in order for the reaction to occur.
Figure 4.2 – Energy changes during the course of an uncatalyzed reaction. Image by Aleia Kim
In Figure 4.3, the activation energy for a catalyzed reaction is overlaid. As you can see, the reactants start at the same energy level (same place on the Y axis) for both catalyzed and uncatalyzed reactions, and the products end at the same energy for both catalyzed and uncatalyzed reactions as well. The catalyzed reaction, however, has a lower energy of activation (dotted line) than the uncatalyzed reaction.
Figure 4.3 – Energy changes during the course of an uncatalyzed reaction (solid green line) and a catalyzed reaction (dotted green line). Image by Aleia Kim
Reversibility
The ratio of reactants to products at equilibrium is a function of the size of the energy difference between the product and reactant states, shown as the different placement on the Y axis on the graphs above. The lower the energy of the products compared to the reactants, the larger the percentage of molecules that will be products at equilibrium.
Since an enzyme lowers the activation energy for a reaction it can speed the reversal of a reaction just as it speeds a reaction in the forward direction. At equilibrium, however, no change in concentration of reactants and products occurs. Thus, enzymes speed the time required to reach equilibrium, but do not affect the ratio of products to reactants at equilibrium.
The ratio of products to reactants can be thought of as a ‘goal’ of sorts for the reaction, though of course molecules and atoms are not living things and do not actually have desires. But it is a state that reactions tend toward. When there are alterations to a system at equilibrium, the reaction will proceed in such a way as to restore that equilibrium ratio.
In particular, in biochemical pathways multiple reactions are linked so that the product of one reaction is the reactant in another. As product is drawn away from the first reaction that system moves from its equilibrium state, and the reaction will proceed to convert additional reactant to product in order to work toward the equilibrium ratio.
Exceptions
A few reactions involve such a large energy release (downward drop on the graphs) as to make the reverse reactions nearly impossible. In these cases the equilibrium ratios are heavily skewed toward products.
They are related to the disappearance of a substrate or product of a reaction. Consider the first reaction below which is catalyzed by the enzyme carbonic anhydrase:
[latex]CO_2 + H_2O \rightleftharpoons H_2CO_3 \rightleftharpoons HCO_3^- + H^+[/latex]
In the forward direction, carbonic acid is produced from water and carbon dioxide. It can either remain intact in the solution or ionize to produce bicarbonate ion and a proton. In the reverse direction, water and carbon dioxide are produced. Carbon dioxide, of course, is a gas and can leave the solution and escape.
When reaction molecules are removed, as they would be if carbon dioxide escaped, the reaction is pulled in the direction of the molecule being lost and reversal cannot occur unless the missing molecule is replaced. In the second reaction occurring on the right, carbonic acid (H2CO3) is “removed” by ionization. This too would limit the reaction going back to carbon dioxide in water. This last type of “removal” is what occurs in metabolic pathways. In this case, the product of one reaction (carbonic acid) is the substrate for the next (formation of bicarbonate and a proton).
In the metabolic pathway of glycolysis, ten reactions are connected in this manner and reversing the process is much more complicated than if just one reaction was being considered.
General mechanisms of action
We have seen now that enzymatically catalyzed reactions are orders of magnitude faster than uncatalyzed and chemical-catalyzed reactions. And that process has been explained using concepts related to energy and equilibrium. But the magic of enzyme catalysis can also be considered from a different point of view: that is, what does an individual enzyme do in order to bring the activation energy barrier on a reaction down?
Changes
One of the remarkable things about catalysts is that they are not reactants in the normal sense: they are not consumed during a reaction. In other words, the catalyst ends up after a reaction just the way it started. Enzymes share this property, although in the midst of the catalytic action, an enzyme is temporarily changed. In fact, it is the ability of an enzyme to change that leads to its incredible efficiency.
These changes may be subtle electronic ones, more significant covalent (bonding) modifications, or structural changes arising from the flexibility inherent in enzymes. These kinds of adjustments are not possible for chemical catalysts such as the metal platinum. Enzyme flexibility allows movement and movement facilitates alteration of electronic environments necessary for catalysis. Enzymes are, thus, much more efficient than rigid chemical catalysts as a result of their abilities to facilitate the changes needed to optimize the catalytic process.
Enzymes are large and complicated molecules, which is the structural basis behind this difference. That complexity also means that a huge variety of enzymes are possible, each with a carefully tuned ability to carry out particular reactions with great efficiency and with great selectivity, working only on certain very specific reactants to convert them to particular products.
Substrate binding and the Active Site
Enzymes have binding sites that not only selectively ‘grab’ a reactant (called a substrate when discussing enzymes), but also place it in a position to be electronically induced to react, either within itself or with another substrate.
The enzyme itself may play a role in the electron shifts or that process may occur as a result of substrates being oriented very close to each other.
The location on an enzyme where substrate binds (the substrate-binding site) is also usually the site of the reaction itself, called the active site.
Enzyme flexibility
As large and complex molecules, enzymes are somewhat flexible and responsive to their local environments. Slight changes in shape, often arising from the binding of the substrate itself, help to optimally position substrates for reaction after they bind.
Figure 4.4 – Substrate binding by methylenetetrahydrofolate reductase
Figure 4.5 – Lysozyme with substrate binding site (blue), active site (red) and bound substrate (black). Wikipedia
Induced fit
These changes in shape are explained, in part, by the Induced Fit Model of Catalysis (Figure 4.6), which illustrates that not only do enzymes change substrates, but that substrates also transiently change enzyme structure. At the end of the catalysis, the enzyme is returned to its original state. This model contrasts with the Lock and Key model, which presents enzymes with a fixed shape that is perfectly matched for binding its substrate(s). Evidence supports the Induced Fit model as a better, truer description of reality.
Enzyme flexibility also plays an important role in control of enzyme activity. Enzymes alternate between the T (tight) state, which is a lower activity state and the R (relaxed) state, which has greater activity.
Figure 4.6 – Fischer’s lock and key model (left) Vs. Koshland’s induced fit model (right). Image by Aleia Kim
Ordered binding
Some enzymes need to bind multiple substrates, for instance if they are catalyzing a reaction where two substrates are covalently linked. For these systems, binding of the first substrate induces structural changes in the enzyme necessary for binding the second substrate. These mechanisms are aligned with the Induced Fit model, where enzymes are able to adjust to the initial binding and thus become more likely to bind the second substrate.
Consider lactate dehydrogenase, the enzyme which catalyzes the reaction below:
[latex]NADH + Pyruvate → Lactate + NAD^+[/latex]
This enzyme requires that NADH must bind prior to the binding of pyruvate. As noted earlier, this is consistent with an induced fit model of catalysis. In this case, binding of the NADH changes the enzyme shape/environment so that pyruvate can bind and without binding of NADH, the substrate cannot access the pyruvate binding site.
Reaction types
Enzyme-catalyzed reactions can be of several types, as shown in Figure 4.7. In one mechanism, called sequential reactions, at some point in the reaction, both substrates will be bound to the enzyme. There are, in turn, two different ways in which this can occur – random and ordered.
Types of Reactions | |
---|---|
Single Substrate – Single Product | A ⇄ B |
Single Substrate – Multiple Products | A ⇄ B + C |
Multiple Substrates – Single Products | A + B ⇄ C |
Multiple Substrates – Multiple Products | A + B ⇄ C + D |
Coenzymes
Organic molecules that assist enzymes and facilitate catalysis are cofactors called coenzymes. The term cofactor is a broad category usually subdivided into inorganic ions and coenzymes. If the coenzyme is very tightly or covalently bound to the enzyme, it is referred to as a prosthetic group. Enzymes without their cofactors are inactive and referred to as apoenzymes. Enzymes containing all of their cofactors are called holoenzymes.
Figure 4.27 – Enzyme cofactors. Image by Aleia Kim
Figure 4.28 – Coenzyme A (CoA)
Some coenzymes are derivatives of vitamins. Vitamins are organic molecules necessary in the diet for normal biochemical function. The chemical structures of vitamins are frameworks that an organism is unable to construct on its own, in adequate quantity. Vitamins as we know them (Vitamin A, vitamin D, etc.) are frequently names that refer to small groups of closely-related chemical structures, any of which can serve the role in our bodies.
Vitamins may undergo chemical modification before playing a particular role in a body. For instance, niacin (Vitamin B3) can be chemically converted to nicotinamide adenine dinucleotide (NAD+), a key molecule in energy metabolism.
Vitamins are frequently incorporated into coenzymes such as this. However not all vitamins serve as coenzymes, and not all coenzymes come from vitamins. The term vitamin is derived from ‘vital amine,’ which is a reference to the need for intake in order to live (‘vital’) and their chemical composition as an amine-another reference which includes many but not all of the chemicals in this group.
Among the cofactors listed in the table Fig. 4.27 are many substances derived from vitamins: every substances named in the list of coenzymes contains a large fragment which comes from one of the B vitamins.
Ribozymes
Nearly all enzymes are proteins. However some RNA molecules are also capable of speeding reactions.
The most famous of these molecules was discovered by Tom Cech in the early 1980s. Cech was puzzled at his inability to find any proteins catalyzing a process his group was studying in a microorganism named Tetrahymena. Ultimately, the catalysis was recognized as coming from RNA itself. Since this discovery, many other examples of catalytic RNAs have been found. Catalytic RNA molecules are known as ribozymes.
359
Figure 4.31 – Cleavage of an RNA by a ribozyme. Wikipedia
Figure 4.32 – Hammerhead ribozyme. Wikipedia
Not unusual
Ribozymes, however, are not rarities of nature. The protein-making ribosomes of cells are essentially giant ribozymes. The 23S rRNA of the prokaryotic ribosome and the 28S rRNA of the eukaryotic ribosome catalyze the formation of peptide bonds.
Ribozymes are also important in our understanding of the evolution of life on Earth. They have been shown to be capable via selection to evolve self-replication. Indeed, ribozymes actually answer a chicken/egg dilemma – which came first, enzymes that do the work of the cell or nucleic acids that carry the information required to produce the enzymes. As both carriers of genetic information and catalysts, ribozymes are likely both the chicken and the egg in the origin of life.